Nanomaterials for Clean Energy
Concept
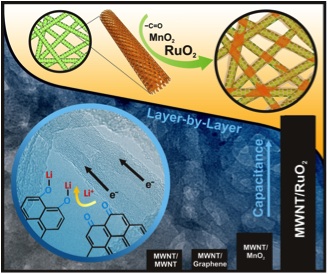
The application landscape for electrochemical energy storage technologies is set to expand rapidly over the next several decades as demand grows in new areas ranging from micro-devices to electrified transportation and clean energy storage and supply. Several unique characteristics of nanostructured electrodes (Fig 1) make them ideal candidates for combining high energy and power at the material level. These advantages include: (i) increased electrochemically active surface areas for charge transfer, (ii) reduction of electronic and ionic transport resistance at smaller diffusion length scales, and (iii) the ability to incorporate high-energy materials into a nanostructured framework capable of sustaining high powers.
Recent advancement of nanostructured electrodes has improved electrochemical performance significantly, providing combined performance of batteries and electrochemical capacitors (ECs). Reducing lithium storage material dimensions down to the nanometer scale can increase the power characteristics of lithium rechargeable batteries, where the time of lithium diffusion accompanying the Faradaic reactions of active particles is decreased. However, nanostructured battery electrodes still have lower power capability than EC electrodes. On the other hand, researchers have increased the gravimetric energy of ECs by utilizing carbon subnanometer pores for ion adsorption or employing the pseudocapacitance of nanostructured transition metal oxides.
Challenges
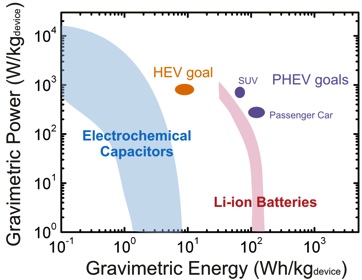
As future applications such as electric transportation and load-leveling will require both high energy and power (Fig 2), a major challenge will be to develop electrode materials that can bridge the performance gap within a single device. By combining high specific surface areas, high electronic conductivity and good mechanical and chemical stability, nanomaterials such as carbon nanotubes, nanofibers, and graphene offer opportunities to develop novel material structures that can potentially reach these goals.
However, these advantages are often difficult to retain due to various difficulties of processing nanomaterials into electrodes or devices, and suitable fabrication techniques are required in order to further develop materials that retain the unique advantages of the nanoscale up to practical electrode thicknesses for next-generation applications. Additionally, exciting opportunities exist for tailoring the surface chemistry and structure of nanomaterials by chemical modification or incorporation into functional composite materials, which can yield new concepts and designs for energy storage and thermoelectric materials.
Research Highlights
Nanostructured electrodes for Li-ion batteries and electrochemical capacitors (ECs)
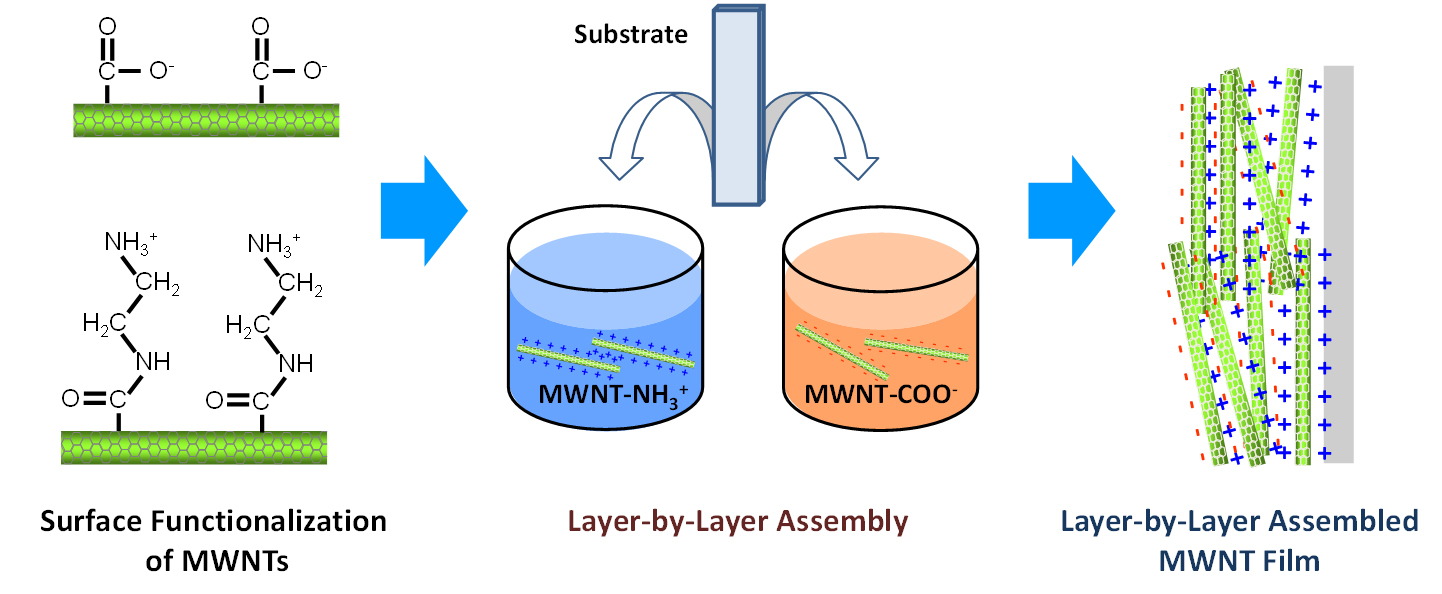
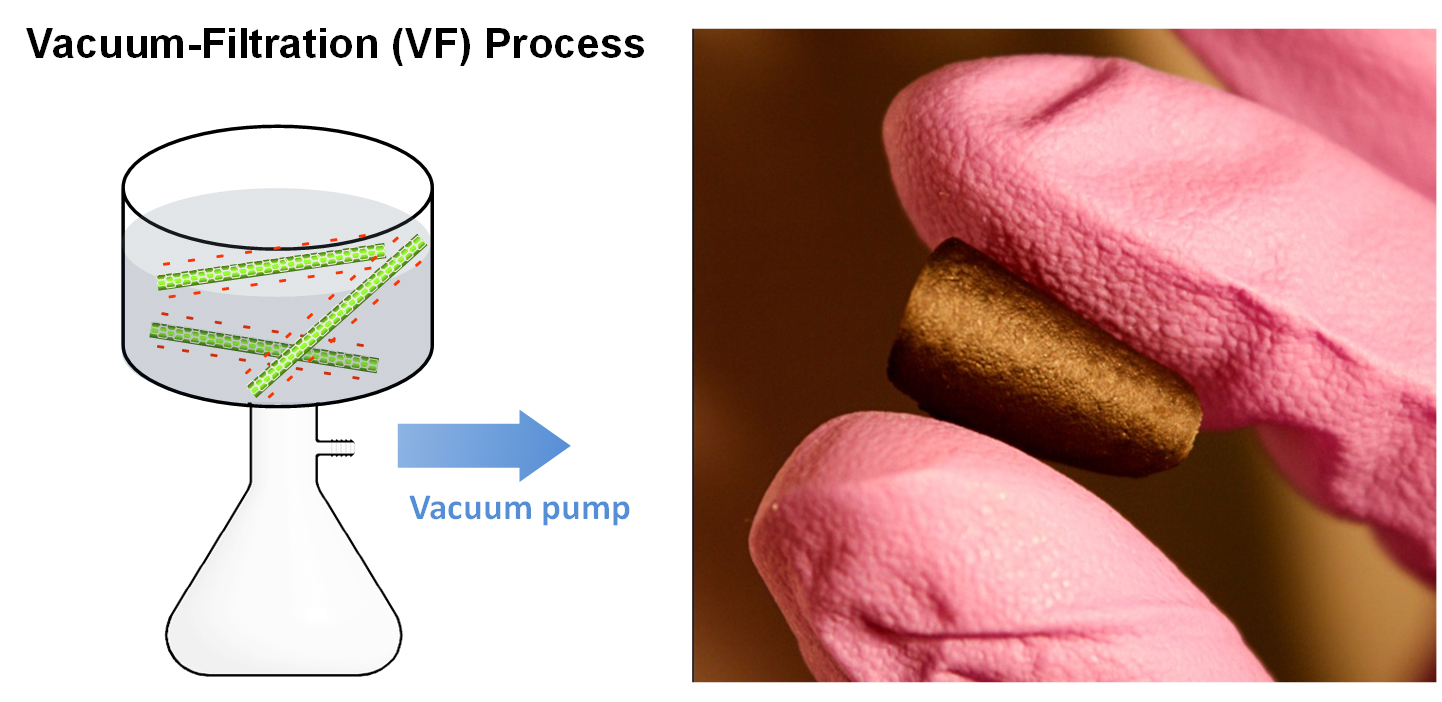
(Bottom) Schematic of vacuum filtration technique and an image of self-standing few-walled carbon nanotube electrodes [Refs. 2, 3]
Novel layer-by-layer (LbL) all-carbon electrodes (Fig 3) can be assembled by repeated, sequential immersion of substrates into aqueous solutions containing positively and negatively charged nano-carbons [1-8]. LbL electrodes have an interpenetrating network structure that can achieve high electronic conductivity, whereas the well-developed porous structure can facilitate fast ion diffusion. We recently reported an alternative approach based on the redox reactions of oxygen functional groups on the surfaces of carbon nanotubes [1, 3, 5]. The electrode, which is several microns thick, can store lithium up to a reversible gravimetric capacity of ~200 mAh/gelectrode while also delivering 100 kW/kgelectrode of power and lifetimes in excess of thousands of cycles, both of which are comparable to electrochemical capacitor electrodes.
A device using the nanotube electrode as the positive electrode and lithium titanium oxide as a negative electrode had gravimetric energy ~5 times higher than conventional electrochemical capacitors, and power delivery ~10 times higher than conventional lithium ion batteries. Finally, binder-free and self-standing carbon nanotube (CNT) electrodes of tens of microns in thickness have been assembled via a vacuum-filtration process of oxidized few-walled CNTs (FWNTs) (Fig 3), with different amounts of oxygen functional groups on FWNTs [3]. These self-standing FWNT electrodes (free of binder/additive and current collector) can provide a high gravimetric energy of ~200 Wh/kg at a high power of ~10 kW/kg, showing promise as the positive electrode for light-weight, high-power lithium batteries.
Nanostructured electrodes for Li-oxygen batteries
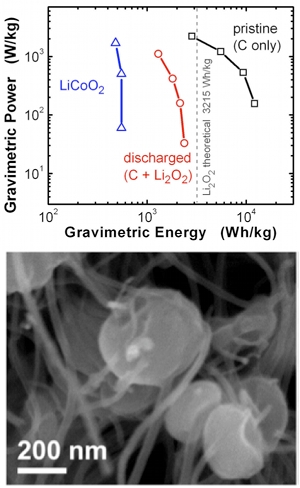
Lithium-oxygen (Li-O2) batteries are promising as next-generation energy storage devices because they offer a theoretical 3-5 times higher gravimetric energy density than existing Li-ion batteries utilizing e.g. LiCoO2 for the positive electrode. This significant advantage in gravimetric energy was recently demonstrated experimentally for the first time[9] (Fig 4) in a Li-O2 cell utilizing aligned carbon nanofibers as the positive electrode, where high porosity (>90% void volume) enabled dense filling of the electrode with the solid discharge product Li2Ox (lithium (per)oxide) that forms and grows during discharge via reduction of O2. By normalizing the performance to the weight of the discharged electrode, when the electrode is in its heaviest state, we demonstrated an energy enhancement of ~4 times compared to LiCoO2, indicating that design of electrode structure is a key consideration for approaching the full potential of Li-O2 batteries.
Perhaps even more interestingly, the unique structure of carbon nanofiber electrodes allowed for clear observation of Li2O2 formation and dissolution during discharge and charge using ex-situ SEM (Fig 4) and TEM studies. Using this approach, we reported a unique Li2O2 particle toroidal structure and showed its detailed evolution during discharge, and its disappearance following charge. This work raises intriguing questions about how Li2O2 nucleation and growth may influence the discharge capacity, round-trip efficiency and cycling capability of Li-O2 batteries, which is not currently understood, and opens up exciting opportunities to develop fundamental understanding of factors currently limiting practical applications of Li-O2 batteries.
Nanostructured thermoelectric materials
Stoichiometric and non-stoichiometric nanostructures in hot pressed Bi2Te2.7Se0.3
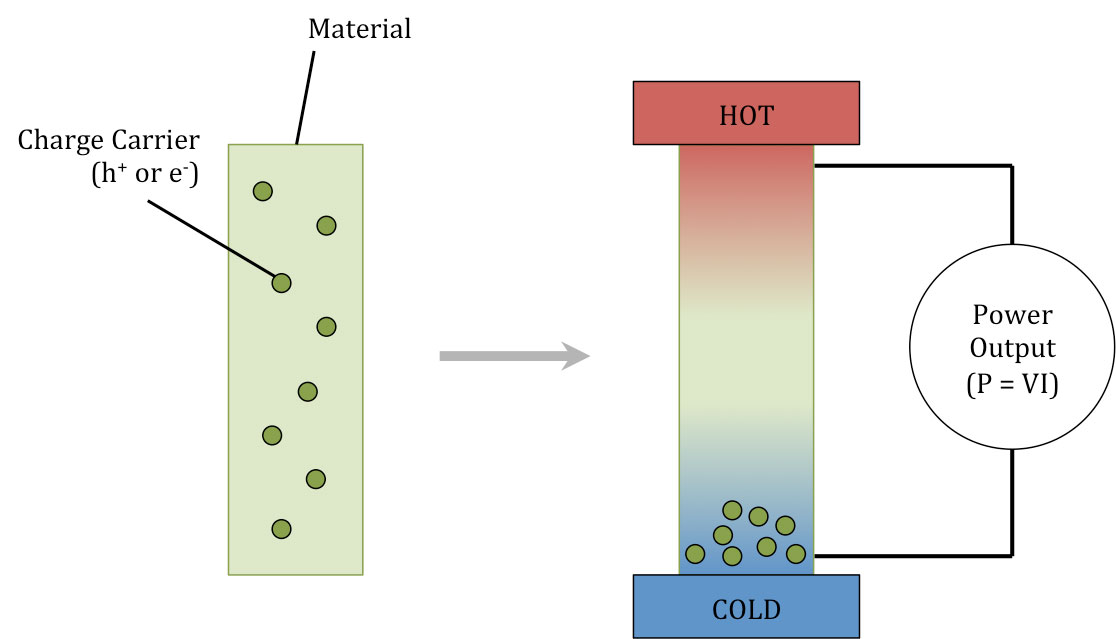
The purpose of our research in thermoelectric materials and devices is to understand nanostructural features in thermoelectric materials and design nanostructures to enhance the figure of merit, which is determined by its electrical conductivity (σ), the Seebeck coefficient (S) and the thermal conductivity (Fig 5). Our recent transmission electron microscopy investigation of as-pressed and re-pressed Bi2Te2.7Se0.3, a promising room temperature thermoelectric material, revealed disordered stoichiometric nanorods and ordered off-stoichiometric nanoparticles.
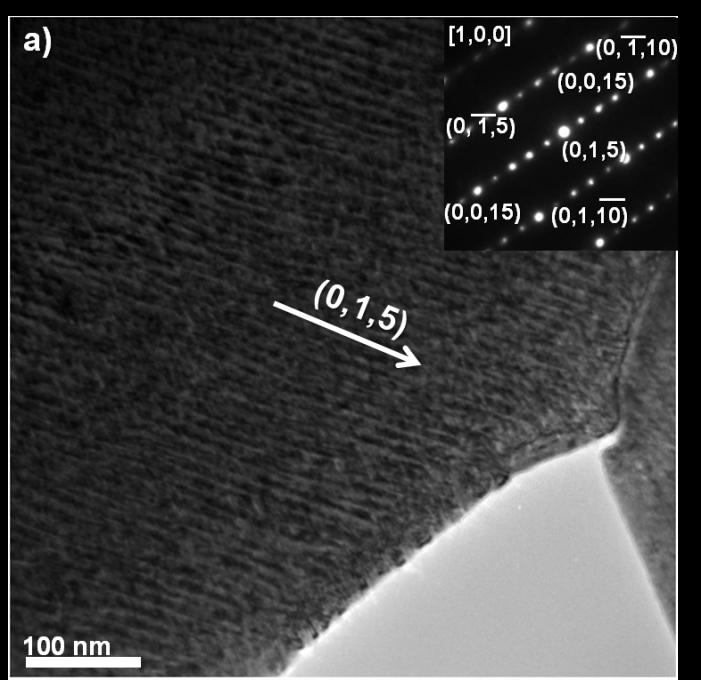
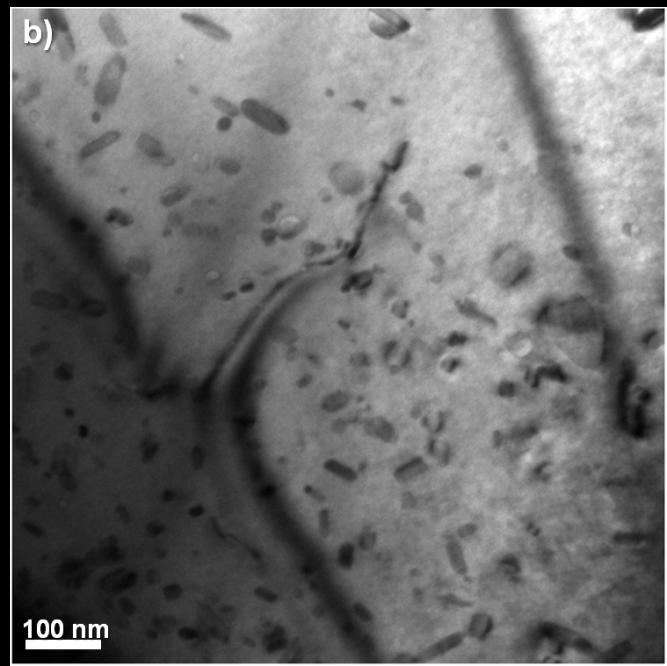
Use of multiple TEM techniques, including bright/dark field diffraction contrast imaging, electron diffraction and high resolution TEM, revealed a network (period ~10nm) of crystalographically aligned disordered/amorphous nanorods with the same composition in every grain (Fig 6a). It is very likely that this nanostructure is responsible for the low thermal conductivity that makes Bi2Te3-system materials such attractive thermoelectric materials. The nanoparticles have Bi2Te3 crystal structures that accommodate Bi-enrichment by inserting additional Te-Bi- and Bi-Bi layers (Fig 6b). Better understanding of these nanoparticles could be used to further reduce thermal conductivity or inhibit grain growth in Bi2Te3-system materials.
References
- Lee, S. W., N. Yabuuchi, B.M. Gallant, S. Chen, B.-S. Kim, P. T. Hammond and Y. Shao-Horn, High-Power Lithium Batteries from Functionalized Carbon Nanotube Electrodes Nature Nanotechnology, 5 (7) 531-537 (July 2010)
- Lee, S. W., B.-S. Kim, S. Chen, Y. Shao-Horn and P.T. Hammond, Layer-by-Layer Assembly of All Carbon Nanotube Ultrathin Films for Electrochemical Applications Journal of the Americal Chemical Society, 131 (2) 671-679 (January 2009)
- Lee, S. W., B. M. Gallant, Y. Lee, N. Yoshida, D. Y. Kim, Y. Yamada, S. Noda, A. Yamada and Y. Shao-Horn, Self-standing Positive Electrodes of Oxidized Few-Walled Carbon Nanotubes for Light-Weight and High-Power Lithium Batteries Energy & Environmental Science 5 (1), 5437 - 5444 (December 2011)
- Hyder, M. N., S. W. Lee, F. C. Cebeci, D. J. Schmidt, Y. Shao-Horn and P.T. Hammond, Layer-by-Layer Assembled Polyaniline Nanofiber/Multiwall Carbon Nanotube Thin Film Electrodes for High-Power and -Energy Storage Applications ACS Nano, 5 (11) 8552–8561 (October 2011)
- Lee, S. W.; B.M. Gallant, H.R. Byon, P.T. Hammond and Y. Shao-Horn, Nanostructured Carbon-Based Electrodes: Bridging the Gap between Thin-Film Lithium-ion Batteries and Electrochemical Capacitors. Perspective Energy & Environmental Science, 4 (6) 1972-1985 (October 2011)
- Byon, H. R., S. W. Lee, S. Chen, P.T. Hammond and Y. Shao-Horn, Thin Films of Carbon Nanotubes and Chemically Reduced Graphenes for Electrochemical Micro-capacitors Carbon, 49 (2) 457-467 (February 2011)
- Kim, B. S.; S. W. Lee, H. Yoon, M. S. Strano, Y. Shao-Horn and P. T. Hammond, Pattern Transfer Printing of Multi-Walled Carbon Nanotube Multilayers and Application in Biosensors, Chemistry of Materials, 22 (16), 4791-4797 (July 2010)
- Lee, S. W.; J. Kim, S. Chen, P. T. Hammond and Y. Shao-Horn, Carbon Nanotube/Manganese Oxide Ultrathin Film Electrodes for Electrochemical Capacitors, ACS Nano, 4 (7), 3889–3896 (June 2010)
- Mitchell, R. R.; B. M. Gallant, C. V. Thompson and Y. Shao-Horn, All-Carbon-Nanofiber Electrodes for High-Energy Rechargeable Li-O2 Batteries Energy & Environmental Science, 2011, 4 (8), 2952-2958 (July 2011)